Rubbish in, rubbish out.
We all know that scientific instruments aren’t magic and can give you data that are only as good as your sample. Often enough in molecular biology, we ask ourselves: well, how good is my sample? Did I perform my assay poorly, or did I feed my assay rubbish?
Insofar as protein samples are concerned, we can answer these questions with a technique called circular dichroism.
This article will take you through the basic principle of circular dichroism and some of its applications. Then, in part 2, we’ll cover its practical aspects and sample preparation using a real-life example.
What Is Circular Dichroism and What Are Its Applications?
Circular dichroism (CD) is a type of spectroscopy that can tell you the type and percentage of secondary structure units in a protein sample.
After a successful CD experiment, you’ll have the answer to these questions:
- Is my protein sample folded?
- What percentages of alpha-helix, beta-sheet, and random coil are in my protein sample?
And because most modern CD spectrometers have variable temperature sample cells:
- How does the secondary structure of my protein sample change with temperature?
- What is the melting point, Tm, of my protein sample?
Granted, answering these questions isn’t going to win you a Nobel Prize because they are quite basic. But it’s the frequency with which they occur that makes them worth addressing.
And perhaps question 1 is so basic, you forget to ask it when things aren’t going right!
For example, you’ve purified a recombinant protein and used it in a series of binding assays. These binding assays have returned a negative result. Do you accept this negative result without knowing if your protein sample is folded?
Let’s instead say that your assays returned a positive result. So, to enrich your data, you’ve mutated a few pertinent residues. Have these mutations altered the structure of your sample? Are you getting these results because of a change in protein structure?
Perhaps you’re farming protein out to give to a collaborator. It’d be a bit of a faux pas to realize way down the line that you’d been providing them with misfolded junk. Better to check that now.
Maybe you’ve attempted to refold your protein and need to check whether or not you’ve been successful.
Or, to give a more particular example, perhaps you’re interested in how the structure of a protein changes to recognize a binding partner. Well, mix them together in a CD spectrometer. It’s way simpler than solving the structure of the complex via crystallography.
An Example Use Case
Still not sold?
At first glance, the above four questions probably sound easy to answer by other methods that are already in your repertoire. Consider the following case, however:
“I work on the novel protease ____. I have purified a recombinant version of ____ and got a band on SDS-PAGE. My gel filtration chromatogram looks dodgy, though, and is far from Gaussian. Also, my activity assay results are garbage. My dream goal is to solve the structure of ____ and implicate it in dreadful disease X.”
Most of us who do our research at the in vitro side of life science have been there.
And if you are unfortunate enough to work on a membrane protein, you probably don’t even have an activity assay. Garbage results are a wistful dream.
So, how much time and energy do you want to invest in pursuing these research goals when you can’t even be sure your sample is folded?
This is where CD comes into the picture. [1] It’s your label-free, substrate-free window into whether or not your purified protein is folded and a starting point from which to begin troubleshooting when things aren’t going to plan.
Pros and Cons of Circular Dichroism
Before we dive into the technical aspects of CD theory, let’s present a quick summary of its pros and cons so you can decide whether CD really is for you (Table 1).
Table 1. The pros and cons of circular dichroism.
Pros | Cons |
Label-free | Low throughput |
Non-destructive (unless you’re heating your sample) | You may not have easy access to a CD spectrometer |
Solution-state measurements | You may need training or somebody to run your sample for you—there’s an extremely brief crash course here? |
Good sample economy. Although this depends, of course, on your particular sample and its quirks | Accurate determination of sample concentration is needed for an accurate breakdown of secondary structure percentage |
The Principle of Circular Dichroism
I hate to break it to you, but CD theory is fundamentally biophysics. It relies on the unusual concept of circularly polarized light and can be a bit hard to wrap your head around.
Essentially, it exploits the unequal absorption of clockwise and anticlockwise circularly polarized light by chiral protein molecules to answer the questions posed above.
Remember, all protein molecules are chiral because the alpha carbon of every constituent amino acid is chiral, except for glycine.
Forgotten what a chiral molecule is? It’s a molecule that cannot be superposed onto its mirror image, just like your hands and feet.
To fully appreciate CD theory and become better scientists, we need to go a bit deeper than this, so that’s what we’ll do.
Stick with me if you don’t fully understand it because I’ll provide a link to a brief video to explain the concept shortly.
A Refresher on How Electromagnetic Waves Move
We’ve all read the textbooks and know that we model electromagnetic photons moving through space as a wave.
The mathematical function that describes these waves is a sine function—the waves are sinusoidal.
If your memory is a bit fuzzy, don’t worry. The four basic properties of photons that we need to appreciate to understand the basis of the CD method are:
- They move (propagate) in a straight line.
- They have an oscillating electric field (labeled E).
- They have an oscillating magnetic field (labeled B).
- The direction of the photon, the electric field, and the magnetic field are all mutually at 90° to each other.
Think of it this way: when a single photon moves directly towards you, head-on, the electric field oscillates vertically up and down, and the magnetic field oscillates horizontally left and right.
When we draw a wave on paper as a sinusoid, the line we draw (the sinusoid) represents the oscillating electric field. The magnetic field is there (it would oscillate in and out of the page), but we just don’t draw it.
Light Polarization and Linearly Polarized Light
Let’s have a think about construction. Take buildings, for example. They are constructed vertically because that’s the most sensible way to do it.
The electric fields of all the photons that are whizzing around us are not all vertical, however. They are not even roughly co-aligned. They are all randomly orientated.
And in many scientific (and recreational) applications, most of these random orientations aren’t useful, so we need to filter them out. In doing so, we leave just photons whose electric field is oscillating up and down, or for that matter, any single plane.
Accept for now that photons can be filtered this way to generate linearly polarized light.
Circularly Polarized Light
“How on earth do you generate circularly polarized light?” I hear you ask. Good question.
We start with linearly polarized light (I’d never waste your time) and then shift its magnetic field one-quarter wavelength backward relative to its electric field.
If this is done, the electric field ends up spiraling around the direction of propagation of the light wave in, yep, a circle. This is called circularly polarized light.
Remember that video I mentioned? It’s here. Imagine the red wave is the electric field, and the blue wave is the magnetic field.
And finally, we can generate circularly polarized light that spirals either clockwise or anticlockwise by shifting the magnetic field forward or backward one-quarter wavelength relative to the electric field.
The Circular Dichroism Experiment
Owing to the unique arrangement of chiral centers in any protein, they will absorb clockwise circularly polarized light better than the anticlockwise light, or vice versa, at any given wavelength.
A CD experiment measures the extent to which the clockwise and anticlockwise circularly polarized light is unequally absorbed by your protein sample after it’s been shone with equal amounts of both.
Here’s the key point—when unequal proportions of clockwise and anticlockwise circularly polarized light are added together, they combine to form a single wave that propagates as an ellipse.
So, because of the unequal absorption of circularly polarized light by chiral protein molecules, and because a CD experiment measures the light that passes from your sample to the detector, a CD experiment measures elliptical light.
And the ellipticity of the light is diagnostic of protein secondary structure. That’s the essence of CD theory.
We’ll illustrate this with a few examples in part 2, but for now, let’s expand on elliptically polarized light with some handy figures.
If the clockwise and anticlockwise circularly polarized photons are all in phase and we add them together, we get back to linearly polarized light (provided that both clockwise and anticlockwise circularly polarized photons also have the same wavelength and amplitude). See Figure 1 for details.
Oh, and “resultant” just means the wave that arises when two or more waves are combined together into a single one.
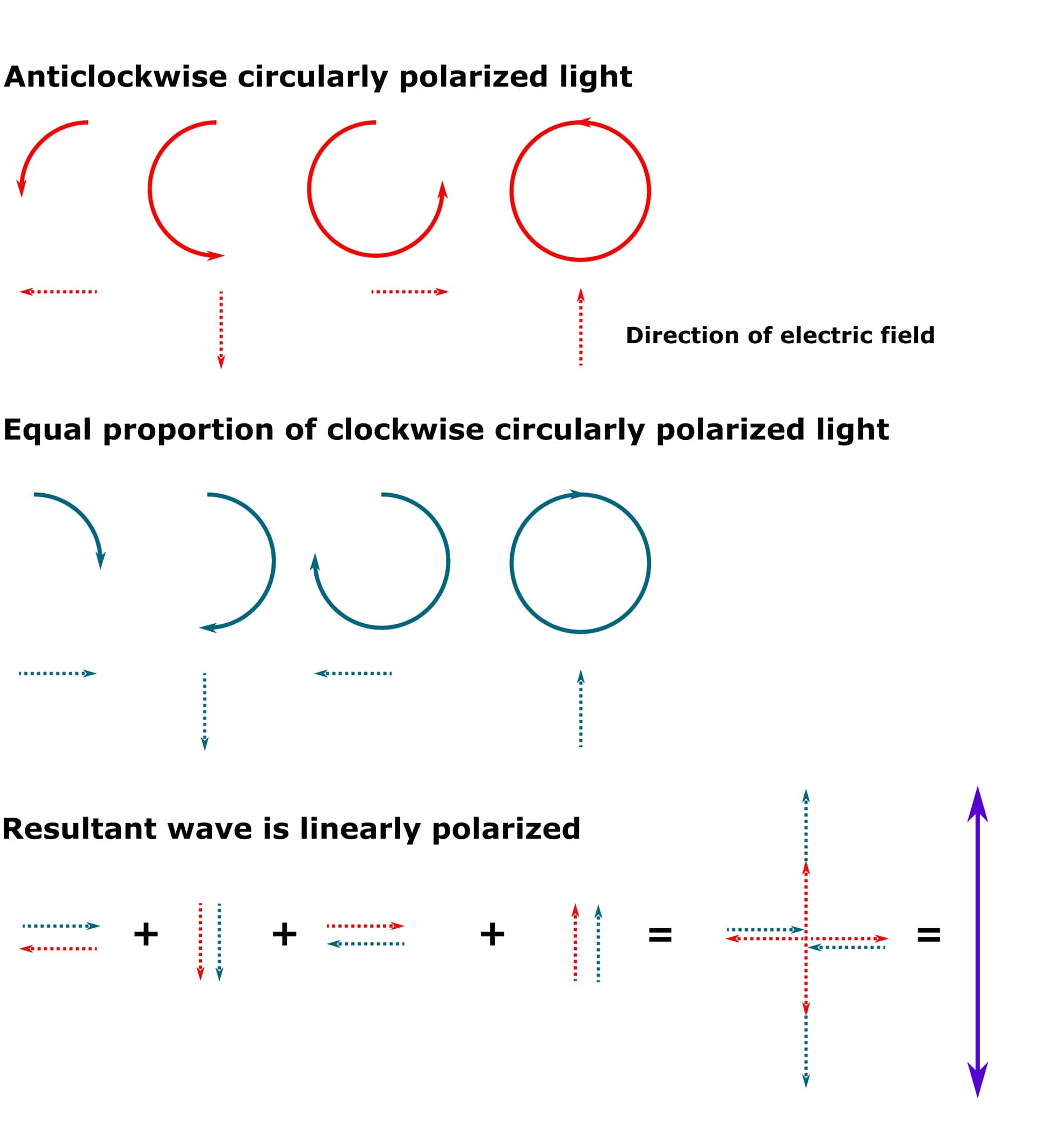
However, when a higher proportion of one direction is absorbed more strongly than the other, as would be the case in a protein sample, the resultant wave is an ellipse. See Figure 2. Note that the smaller circle for the clockwise circularly polarized light is taken to represent a higher degree of absorption by the chiral centers in the sample. However, it could also represent a wave with a lower amplitude (but the same wavelength) than the anticlockwise circularly polarized light. The result would be the same.
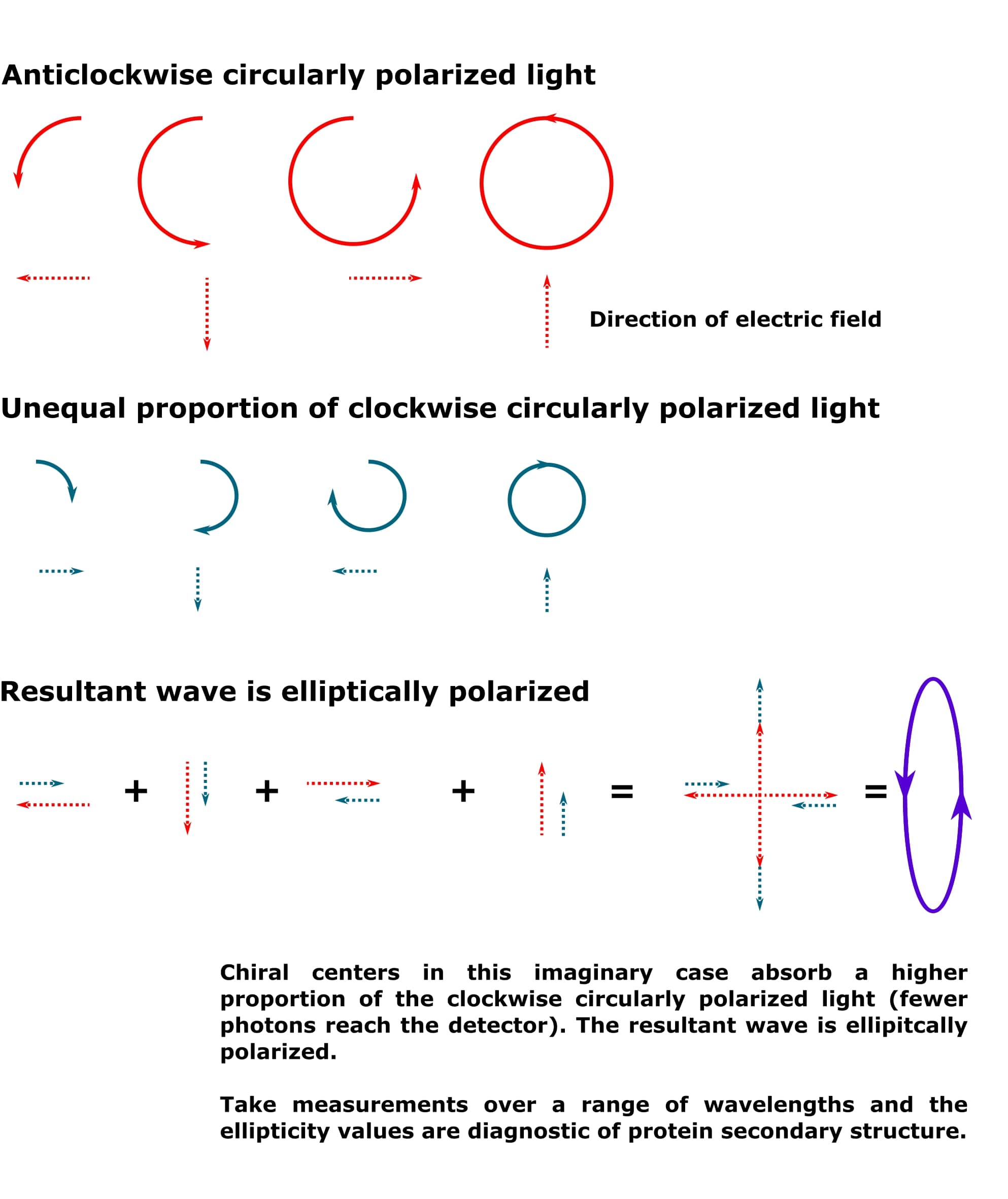
Because the chiral centers in a protein will absorb clockwise and anticlockwise circularly polarized light to different extents, the resultant light that passes from your sample to the detector is always elliptically polarized.
And the conformation of the chiral alpha carbons in a protein accurately describes its secondary structure.
Chiral centers are crucial to the circular dichroism experiment, therefore.
The extent to which chiral centers unequally absorb clockwise and anticlockwise light depends on the following three factors:
- Which enantiomer (L- or D-form) is present.
- The conformation of the chiral center.
- The local chemical environment of the chiral center.
We can control the chemical environment of the chiral centers by putting our protein sample in a well-defined buffer solution.
So, the degree of ellipticity of the resultant wave that arises after our sample has been shot with both clockwise and anticlockwise light is diagnostic of its secondary structure elements.
And in a CD experiment, ellipticity is measured over a range of wavelengths in the UV region, usually, \lambda = 180–260 nm.
If you’re still with me, you deserve a sticker!
Hang around for part 2, where we’ll move on from CD theory and get into the fun (easier) stuff. This will get you ready to go and collect a CD spectrum from your sample.
References
- Greenfield NJ (2006) Using circular dichroism spectra to estimate protein secondary structure. Nat Protoc 1:2876–90